
Research Areas
Image-Guided Ultrasound Therapeutics Laboratories Research Areas
The use of ultrasound and microbubbles as an effective adjuvant to thrombolytics has been demonstrated in vitro, ex vivo, and in vivo at the Image-guided Ultrasound Therapeutics Laboratories (IgUTL) (Bader et al. 2016). Studies at IgUTL have focused on harnessing the stable cavitation mechanism to improve stroke treatment with sub-megahertz (120-220 kHz), unfocused ultrasound. At these frequencies, minimal transcranial distortion of the ultrasound field occurs, only external landmarks are required to ensure homogenous insonation of the thrombus with the unfocused field (Bouchoux et al. 2014), and the FDA-approved microbubble Definity® rapidly coalesces to resonant size (Bader et al. 2015a). Microbubble coalescence allows for stable cavitation, a key mechanical mechanism through which ultrasound enhances thrombolysis.
Thrombus composition varies between patients based on the conditions in which thrombi are formed, giving rise to thrombi with varying susceptibility to the FDA-approved thrombolytic recombinant tissue-type plasminogen activator (rt-PA). Stable cavitation best enhances rt-PA activity in less retracted clots, while stiffer, more retracted thrombi may require other forms of cavitation activity to enhance thrombolysis (Bader et al. 2015b). Furthermore, targeted delivery of rt-PA may decrease the adverse reaction rate associated with the drug. Echogenic liposomes (ELIP) containing gas microbubbles have been developed as a vector for therapeutic drugs. Thrombolytic-loaded liposomes, termed t-ELIP, target fibrin, and can be acoustically activated for localized drug delivery. Encapsulation of rt-PA may reduce systemic toxicity compared to direct intravenous injection of this potent thrombolytic, while ultrasound insonation of the gas microbubbles within t-ELIP can potentially release the rt-PA locally and incite stable cavitation. Encapsulation of octafluoropropane gas in t-ELIP has shown promise to increase both stable cavitation activity and thrombolytic efficacy compared to rt-PA alone (Shekhar et al. 2016).
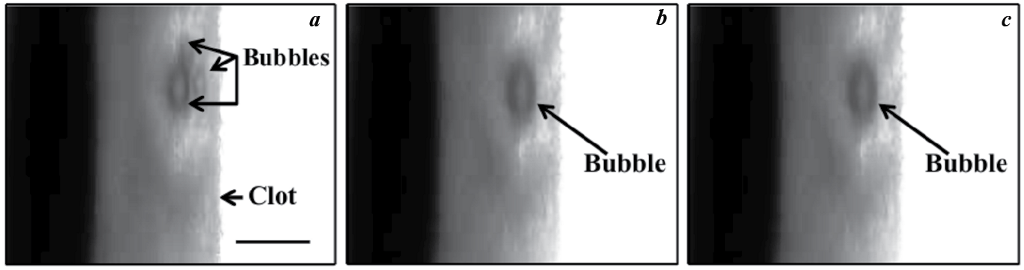
Figure. Coalescence and translation of bubble. (a) Prior to insonation, three bubbles are visible on the clot. (b) The three bubbles coalesce within 400 ms after ultrasound exposure. (c) The resultant bubble translates after remaining stationary for 3.5 s. The surrounding fluid contains recombinant tissue-type plasminogen activator (0.32 μg/mL), and Definity® (2 μL/mL). The bubble appears distorted due to the long exposure time of the camera (16 ms) compared to the acoustic period (8.33 μs). The scale bar in panel a is 100 μm. Images taken from Bader et al. 2015a.
The Image guided Ultrasound Therapeutics Labs at the University of Cincinnati are currently developing a novel transcranial ultrasound thrombolysis system (TUTS). With TUTS, an ultrasonic transducer is held against the temporal bone; energy radiates from the transducer, through the bone, and into the brain. It is hoped that application of ultrasound via TUTS will permit the usage of lower dosages of rt-PA than are currently used clinically, yet resulting in increased thrombolysis with reduced risks of hemorrhage. The benefits of the TUTS system potentially include an increased number of stroke survivors, improved long-term prognosis and reduced health care costs.
References
- Bader KB, Bouchoux G, Holland CK (2016) Sonothrombolysis. In: Ther. Modalities. pp 339–362. doi:10.1007/978-3-319-22536-4_19
- Bader KB, Gruber MJ, Holland CK (2015a) Shaken and Stirred: Mechanisms of Ultrasound-Enhanced Thrombolysis. UMB 41:187–196. doi:10.1016/j.ultrasmedbio.2014.08.018
- Bader KB, Haworth KJ, Holland CK, et al (2015b) Fibrin-targeted echogenic liposomes for localized ablation of thrombi with histotripsy pulses. In: Acoustical Society of America. Jacksonville, pp 1–2. doi:10.1121/1.4933777
- Bouchoux G, Shivashankar R, Abruzzo TA, Holland CK (2014) In silico study of low-frequency transcranial ultrasound fields in acute ischemic stroke patients. UMB 40:1154–1166. doi:10.1016/j.ultrasmedbio.2013.12.025
- Shekhar H, Huang S, Peng T, et al (2016) Thrombolytic efficacy of echogenic liposomes that co-encapsulate rt-PA and octafluoropropane gas. The Journal of the acoustical Society of America 139:2093–2093. doi:10.1121/1.4950212
Bioactive gases can cross biological barriers readily and produce therapeutic effects. Until recently, bioactive gas delivery has been hampered by lack of suitable administration methods and possibility of adverse off-target effects. Researchers at IgUTL in collaboration with colleagues at University of Texas health sciences center Houston (UTHSC), are advancing ultrasound-triggered bioactive gas delivery for cardiovascular and neurological applications. In particular, we have contributed towards advancing ultrasound-triggered delivery of Nitric Oxide and Xenon. Nitric oxide (NO) has potent vasodialatory, anti-inflammatory, antithrombotic, antiproliferative, neuroprotective, and anti-atherogenic properties (Klegerman et al. 2010). Xenon is a neuroprotectant that easily crosses the blood-brain barrier, and has demonstrated efficacy for alleviating acute neuronal injury (Britton et al. 2010).
We have developed and characterized liposomal formulations that are suitable for bioactive gas encapsulation, and demonstrated the feasibility of ultrasound-triggered bioactive gas delivery in ex vivo and in vivo models (Klegerman et al. 2010, Britton et al. 2010, Sutton et al. 2014, Huang et al. 2009, Kim et al. 2014). NO-loaded echogenic liposomes (NO-ELIP) serve as an ultrasound-triggered delivery platform for site-specific delivery of NO (Sutton et al. 2014, Kim et al. 2014). In addition to being a convenient administration vehicle, encapsulating NO within a lipid shell shields this highly reactive gas from hemoglobin scavenging in the bloodstream. Using an ex vivo porcine carotid model in the presence of hemoglobin, we have already demonstrated the feasibility of NO delivery (Sutton et al. 2014). The delivery of NO in response to ultrasound was confirmed by arterial vasorelaxation (Fig. 1) with a similar effect to sodium nitroprusside. The delivery of NO-ELIP to rabbit coronary arteries to prevent neointimal hyperplasia due to angioplasty balloon injury was also reported (Huang et al. 2009). In addition, our work has also elucidated the neuroprotective effects of Xenon released from xenon-loaded liposomes using cell culture, and an in vivo rat model of middle cerebral artery ischemia/reperfusion (Britton et al. 2010). Cumulative evidence from our research suggests that ultrasound-mediated bioactive gas delivery could emerge as a powerful tool for therapeutic mediation in a range of cardiovascular and cerebrovascular pathologies.
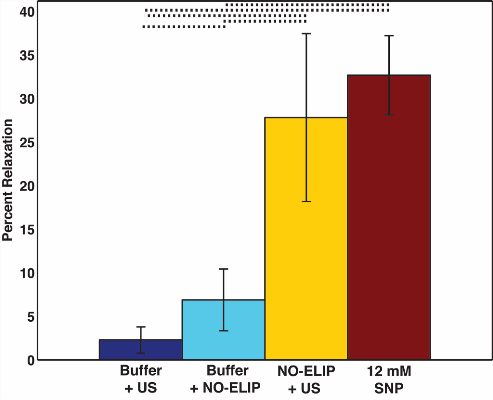
Figure. The feasibility of producing vasorelaxation with NO-ELIP. The percentage vasorelaxation of porcine carotid arteries is shown for different treatments. Sodium nitroprusside (SNP), a NO donor, served as a positive control. Dotted lines indicate statistical significance (N=7).
Other therapeutic agents of interest include anti-inflammatory drugs (rosiglitazone),thrombolytics (recombinant tissue plasminogen activator), anti-angiogenesis (bevacizumab), vasoactive gases (nitric oxide and xenon), and anti-inflammatory genes (eNOS). Experiments at the Image-guided ultrasound therapeutics labs are focused on the development of optimal ultrasound parameters for therapeutic delivery and determination of the efficacy of therapeutic release to arrest atheroma progression.
References
- Klegerman ME, Wassler M, Huang SL, Zou Y, Kim H, Shelat HS, Holland CK, Geng YJ, McPherson DD. Liposomal modular complexes for simultaneous targeted delivery of bioactive gases and therapeutics. J Control Release. 2010, 19;142(3):326-31. doi:10.1016/j.jconrel.2009.10.037
- Britton GL, Kim H, Kee PH, Aronowski J, Holland CK, McPherson DD, Huang SL. In vivo therapeutic gas delivery for neuroprotection with echogenic liposomes. Circulation. 2010, 122(16):1578-87. doi: 10.1161/CIRCULATIONAHA.109.879338
- Sutton JT, Raymond JL, Verleye MC, Pyne-Geithman GJ, Holland CK. Pulsed ultrasound enhances the delivery of nitric oxide from bubble liposomes to ex vivo porcine carotid tissue. Int J Nanomedicine. 2014, 6;9:4671-83. doi:10.2147/IJN.S63850
- Huang SL, Kee PH, Kim H, Moody MR, Chrzanowski SM, Macdonald RC, McPherson DD. Nitric oxide-loaded echogenic liposomes for nitric oxide delivery and inhibition of intimal hyperplasia. J Am Coll Cardiol. 2009, 11;54(7):652-9. doi:10.1016/j.jacc.2009.04.039
- Kim H, Britton GL, Peng T, Holland CK, McPherson DD, Huang SL. Nitric oxide-loaded echogenic liposomes for treatment of vasospasm following subarachnoid hemorrhage. Int J Nanomedicine. 2014; 9: 155–165. doi:10.2147/IJN.S48856
Acoustic droplet vaporization (ADV) is the process whereby liquid perfluorocarbon droplets undergo a phase transition into gas microbubbles when insonified with ultrasound above a pressure threshold. The volumetric expansion of the perfluorocarbon during the phase transition results in microbubbles that are highly undersaturated with non-perfluorocarbon gas molecules relative to the surrounding fluid. In particular, the relative oxygen saturation in the microbubble could be three orders of magnitude lower than that in the surrounding fluid. Thus, the microbubbles formed via ADV act as potent sinks that scavenge dissolved gases from surrounding fluids. We have demonstrated the ability to scavenge dissolved oxygen from both saline and from porcine whole blood using an in vitro flow model. A numerical model based on the mechanisms concentration gradients has been developed and can be used to predict the expected decrease in dissolved oxygen (Radhakrishnan et al. 2016). This numerical model indicates that the key factor in scavenging oxygen is the total volume of perfluorocarbon that is converted from a liquid to a gas. To translate the effect in vivo, we have developed a differential centrifugation protocol to size-isolate droplets that transition most efficiently, while also removing droplets that are too large to pass through the vasculature (Mercado et al. 2016).
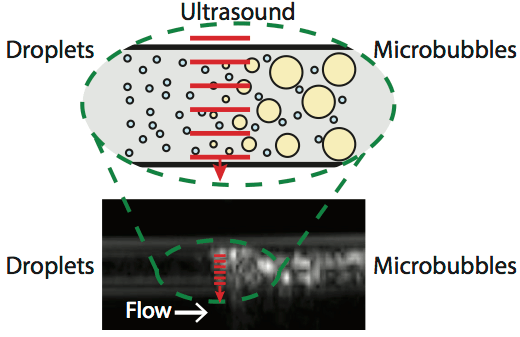
Figure 1. Acoustic droplet vaporization. The top portion of the figure is a magnified schematic of the ADV process, shown on a B-mode image in the lower part of the figure. Droplets are convected through a flow tube from left to right. The droplets appear hypoechoic (dark) in the lumen. After ADV, the resulting microbubbles are hyperechoic (bright). The droplets (small blue circles) do not phase transition into microbubbles (yellow circles) until they are exposed to ultrasound.
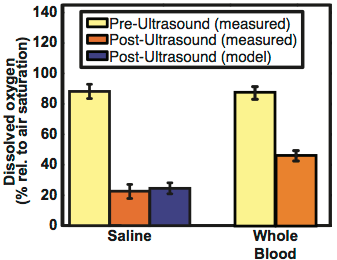
Figure 2. Dissolved oxygen scavenging. The dissolved oxygen of fluids containing perfluoropentane droplets was measured before and after ultrasound exposure in a flow phantom. Approximately, a 75% and 50% reduction in dissolved oxygen was measured after ultrasound exposure in saline and whole blood, respectively.
References
- Mercado KP, Radhakrishnan K, Stewart K, Snider L, Ryan D, Haworth KJ. Size-isolation of ultrasound-mediated phase change perfluorocarbon droplets using differential centrifugation. J Acoust Soc Am Acoustical Society of America, 2016;139:EL142–EL148. doi:10.1121/1.4946831
- Radhakrishnan K, Holland CK, Haworth KJ. Scavenging dissolved oxygen via acoustic droplet vaporization. Ultrason Sonochem 2016;31:394–403. doi:10.1016/j.ultsonch.2016.01.019
As demonstrated at IgUTL and other laboratories around the world, cavitation is associated with a several bioeffects, including sonothrombolysis, HIFU thermal ablation, sonoporation, and drug and gene delivery. The microbubble activities that induce these bioeffects also produce characteristic cavitation emissions, which can be detected using single-element transducers. Single-element transducers provide good spatial sensitivity or specificity for the detection of cavitation emissions, but not both. Passive cavitation imaging is an array-based approach to processing cavitation emissions that have excellent spatial sensitivity and specificity. Array-based passive cavitation imaging can provide superior signal-to-noise as compared to single-element transducers by destructive interference of incoherent noise across the individual elements in an array. Through our experiments and analysis, we have demonstrated that the resolution of passive cavitation imaging is diffraction dependent (i.e., primarily depends on the frequency content of the signals being beamformed and the passive array element spacing and geometry). Therefore the image resolution does not depend on pulse shape or length, in contrast to standard B-mode imaging.
At IgUTL, we have demonstrated that frequency-domain delay-and-sum passive cavitation imaging can accurately map different types of cavitation activity based on the frequency content of cavitation emissions (Haworth et al. 2012). The frequency-domain approach can be substantially faster than time-domain delay-and-sum algorithms due to the inherent frequency-selectivity of the approach. Studies at IgUTL have developed and implemented passive cavitation imaging in vitro, ex vivo, and in vivo on clinical and research ultrasound scanners. We have also applied passive cavitation imaging to the study of bubble dynamics (Radhakrishnan et al. 2015), ultrasound-mediated drug delivery (Haworth et al. 2016), and high-intensity focused ultrasound thermal (Haworth et al. 2015) and mechanical ablation (Bader et al. 2015). These results show that passive cavitation imaging has the potential to be a powerful tool for monitoring and controlling cavitation-based therapies.

Figure. Passive cavitation images of subharmonic emissions overlaid on ultrasound b-mode images of echogenic liposomes flowing (left to right) through a vessel phantom. Cavitation of the echogenic liposomes is induced with a clinical scanner in spectral Doppler mode (MI = 0.8). Loss of echogenicity in the b-mode images occurs at the same spatial location as subharmonic emissions in the passive cavitation images.
References
- Bader KB, Haworth KJ, Maxwell AD, Peng T, McPherson DD, Holland CK. Fibrin-targeted echogenic liposomes for localized ablation of thrombi with histotripsy pulses. J Acoust Soc Am Acoustical Society of America, 2015;138:1820-1820. doi:10.1121/1.4933777
- Haworth KJ, Mast TD, Radhakrishnan K, Burgess MT, Kopechek JA, Huang S-L, McPherson DD, Holland CK. Passive imaging with pulsed ultrasound insonations. J Acoust Soc Am Acoustical Society of America, 2012;132:544-553. doi:10.1121/1.4728230
- Haworth KJ, Raymond JL, Radhakrishnan K, Moody MR, Huang S-L, Peng T, Shekhar H, Klegerman ME, Kim H, McPherson DD, Holland CK. Trans-Stent B-Mode Ultrasound And Passive Cavitation Imaging. Ultrasound Med Biol 2016;42:518-527. doi:10.1016/j.ultrasmedbio.2015.08.014
- Haworth KJ, Salgaonkar VA, Corregan NM, Holland CK, Mast TD. Using Passive Cavitation Images to Classify High-Intensity Focused Ultrasound Lesions. Ultrasound Med Biol 2015;41:2420-2434. doi:10.1016/j.ultrasmedbio.2015.04.025
- Radhakrishnan K, Haworth KJ, Peng T, McPherson DD, Holland CK. Loss of echogenicity and onset of cavitation from echogenic liposomes: pulse repetition frequency independence. Ultrasound Med Biol 2015;41:208-221. doi:10.1016/j.ultrasmedbio.2014.08.021
Based on observations that tissue heating coincides with substantial, rapid fluctuations in echo signals, we have developed echo decorrelation imaging for thermal ablation monitoring (Mast 2008; Subramanian 2014; Fosnight 2014). Echo decorrelation imaging is a pulse-echo imaging method that quantifies and maps ultrasound echo changes on millisecond time scales throughout an ultrasound image plane, quantifying echo changes as a function of position. Our analysis has shown that echo decorrelation comprises a combination of intrinsic tissue-medium changes with motion and noise effects, leading to methods for removing motion and noise artifacts as well as quantitative characterization of tissue structural changes (Hooi 2015).
We are currently investigating echo decorrelation imaging as a method for monitoring and control of thermal cancer therapy, including radiofrequency ablation (RFA), the primary clinical treatment modality for unresectable liver cancer and other tumors of soft tissue and bone. Echo decorrelation imaging can also monitor other thermal ablation methods, including high-intensity focused ultrasound ablation. Our research to date has confirmed the ability of echo decorrelation imaging to predict heat-induced tissue coagulation both in vitro and in vivo, caused by RFA (Mast 2008; Subramanian 2014; Subramanian 2016) as well as focused and unfocused ultrasound ablation of VX2 tumors in rabbit liver (Subramanian 2014; Fosnight 2014). Ongoing efforts include study of closed-loop thermal ablation control based on real-time echo decorrelation feedback.

Figure. In-vivo ultrasound echo decorrelation imaging of radiofrequency ablation in porcine liver. (a) Hybrid B-scan/echo decorrelation image of RFA from a 7 MHz, 192-element linear array probe. (b) Corresponding echo decorrelation image after correction for motion and noise artifacts. (c) Comparison with segmented, registered tissue histology, showing good agreement between regions of elevated echo decorrelation and ablation. (d) Receiver Operating Characteristic curves for prediction of liver ablation by echo decorrelation imaging and integrated backscatter imaging from 5 treatments, showing significantly better ablation prediction using echo decorrelation compared to integrated backscatter.
References
- Subramanian SE, Schmidt DT, Rao MB, Mast TD. Dependence of ultrasound echo decorrelation on local tissue temperature during ex vivo radiofrequency ablation. Phys Med Biol 61, 2356–2371 (2016). doi:10.1088/0031-9155/61/6/2356
- Hooi FM, Nagle S, Subramanian S, Mast TD. Analysis of tissue changes, measurement system effects, and motion artifacts in echo decorrelation imaging. J Acoust Soc Am 37, 585–597 (2015). doi:10.1121/1.4906580
- Fosnight TR, Hooi FM, Keil RD, Subramanian S, Barthe PG, Wang Y, Ren X, Ahmad S, Mast TD. Motion-corrected echo decorrelation imaging of in vivo focused and bulk ultrasound ablation in a rabbit liver cancer model. IEEE Ultrasonics Symposium Proceedings (2014). doi:10.1109/ULTSYM.2014.0538
- Subramanian S, Rudich SM, Karunakaran CP, Rao MB, Mast TD. In vivo thermal ablation monitoring using ultrasound echo decorrelation imaging. Ultras Med Biol 40, 102–114 (2014). doi:10.1016/j.ultrasmedbio.2013.09.007
- Mast TD, Pucke DP, Subramanian SE, Bowlus WJ, Rudich SM, Buell JF. Ultrasonic monitoring of in vitro radiofrequency ablation by echo decorrelation imaging. J Ultrasound Med 27, 1685–1697 (2008). PMID: 19022994
Expand all
Collapse all
Lab Facilities
- Ultrasound Research Lab - CVC 3940
- Ultrasound Microscopy and Imaging Processing Core - CVC 3947
- Ultrasound Imaging Lab - CVC 3947
- Molecular Imaging and Biomolecular Assessment Lab - CVC 3942
- Tissue Culture Lab - CVC 3945
- Biomedical Ultrasonics and Cavitation Laboratory - CVC 3972 (Kevin Haworth, Ph.D.)
- Biomedical Acoustics Lab - CVC 3960 (T. Douglas Mast, Ph.D.)
Contact Us
Image-GuidedUltrasound Therapeutics Laboratories
Cardiovascular Center
University of Cincinnati 3935
231 Albert Sabin Way
PO Box 670769
Cincinnati, OH 45267-0586
Mail Location: 0586
Phone: 513-558-5675
Email: Christy Holland